FIRST JOINT DETECTION OF GRAVITATIONAL AND ELECTROMAGNETIC EMISSIONS FROM A NEUTRON STARS MERGER
On August 17th, 2107, an exceptional event has mobilized the international astronomical community: the fusion of 2 neutron stars was detected both by gravitationnal waves (GW) and by the subsequent light emission, a short burst in gamma rays, and a kilonova in visible and near infrared light. This is the beginning of a new, multi-messenger astronomy : gravitational and luminous. These remarkable observations largely confirm the theoretical scenario which was considered for these rare, cataclysmic events. By their earlier theoretical work, and their participation in the observations of this event, researchers at the IAP have significantly contributed to this discovery, whose first results were made public on Monday Oct. 16th, 2017.
On August 17th, 2017, at 12:41 UT, the two LIGO interferometers in the USA detect a gravitational wave signal, that is soon interpreted as originating from the fusion of two neutron stars. Less than two seconds later, a gamma-ray burst is detected by the Fermi satellite and this information is immediately relayed to the astronomical community via a complex and rapid procedure using US communication satellites. About forty minutes later, the collaboration between LIGO and the third interferometer Virgo, located in Italy, announces their joint detection, emphasizing the temporal coincidence with the gamma-ray burst and that the gravitational event corresponds to the coalescence of two neutron stars. Many observatories then start to search for other possible emissions associated with this event. To this end, they have at their disposal the results of the joint analysis of the data from the three interferometers LIGO-Virgo: they are provided a few hours after the initial announcement, and gives an estimate of the distance, of the order of 40 megaparsecs (about 130 million light-years), as well as the approximate location of the source on the sky, within an area of about 30 square degrees (Figure 1). It is the first time that such a precision is available for a gravitational wave emission.
Figure 1 : Error boxes of the various instruments, highlighting the crucial contribution of Virgo in reducing the uncertainty on the sky location of the source: LIGO alone is shown in light green, LIGO + Virgo in dark green. For comparison, the larger error boxes from the gamma-ray detections are shown in blue: Fermi in dark blue, Integral in light blue. The intersection of these various error boxes made it possible to reduce the zone where they searched for the optical emission. The optical image in the upper right shows the lenticular galaxy NGC 4993, in the halo of which the kilonova has been detected (marked by perpendicular tick marks to the upper left of the galaxy center). The lower right optical image shows that no star was visible at that position 20 days before the fusion, while in the upper image, the kilonova is about as bright as the other stars in the field, which belong to our Milky Way, and are therefore much closer to us (Source: Abbott et al. 2017, The Astrophysical Journal Letters).
It is crucial to proceed very fast to identify “counterparts”, that is other types of emission arising from the same object. Those originating from such a phenomenon are expected to fade rapidly and may soon become undetectable. The distance information provided by the gravitational wave allows several observatories to optimize their search, by concentrating on the few tens of galaxies located in the error box.
It is about ten hours after the first alert, once nightfall made it possible to start the observations in Chile, that the intensive search succeeds: a new point source, previously undetected, is first identified by the “Swope Supernovae Survey” collaboration, with the 1m telescope at Las Campanas, in the NGC 4993 galaxy located at the distance predicted by the gravitational wave emission. This “transient” event is named SSS 17a. Five other groups of observers detect the same source, independently, and during the same night, in other observatories.
Once this optical counterpart is identified, further observations using many telescopes from the ground and from space are performed during the following hours and days, in a rare example of nationwide collaboration. These observations made it possible to identify the optical counterpart as a kilonova[1] and to study its evolution in detail.
The gravitational wave emission
The observation, in an interferometer such as those of LIGO-Virgo, of a signal whose frequency is rapidly increasing and then disappears, is the signature of the arrival on Earth of gravitational waves generated by the coalescence of two compact objects. In the case of the event of August, 17th 2017, the signal is detected during 100 seconds in the two LIGO interferometers. Figure 2 shows the last 30 seconds in one of the interferometers.
Figure 2 : Diagram produced by the LIGO-Hanford interferometer (Washington state), showing the increase of the frequency of the gravitational wave train with time, indicated as the line with higher density (green-yellow ), running from bottom to top as time increases (toward the right). This signal is produced while the two neutron stars get closer to each other following a spiraling orbit. The graph shows only the last 30 seconds of the detection. The increasing deflection of the curve toward the top corresponds to the final phase, during which the two neutron stars spiral in faster and faster ending up n the merger itself, also called « coalescence », here taken to be the 0 instant (Source : Abbott et al. 2017, Physical Review Letters).
Gravitational waves are ripples of space-time predicted by the theory of general relativity, which propagate at the speed of light and are generated by extremely rapid, i.e. “relativistic” (with velocities close to the speed of light), displacements of large amounts of matter. Binary systems[2] of compact objects, such as neutron stars[3], generate gravitational waves at frequencies proportional to the orbital frequency of the motion of the two stars around each other. The wave takes away a part of the system energy, and as a result, the two stars get gradually closer and closer, following a “spiraling” orbit whose orbital frequency (i.e. the number of orbits traveled by the stars in one second) is increasing, resulting in the frequency rise of the gravitational wave signal ((Figure 2(). Then the stars finally merge: this is the so-called coalescence.
In the case of neutron stars, the LIGO and Virgo detectors are mostly sensitive to the inspiraling phase of the two stars, prior to their final merger. Thus the gravitational waves are observed before the electromagnetic emissions which are produced during and after the coalescence. At the instant of the merger, the equivalent of a small fraction of the mass of the system (a few hundredths of the mass of the Sun) is taken from the gravitational binding energy of the two stars and transferred as energy to the gravitational waves, according to the famous formula E = mc2 (the energy E equals the mass m times the square of the speed of light c).
Observing the gravitational wave during the inspiral phase allows the researchers to measure the individual masses of the two neutron stars and also their “spin” (i.e. the angular momentum which results from the combination of their mass and their rotation velocity). A preliminary analysis[4] yields masses between 1.36 and 1.60 solar masses for the first star, and between 1.17 and 1.36 for the second one (but a particular combination of the two masses, called the “chirp” mass, is determined with a higher precision). These ranges of masses for the two stars are consistent with a binary system of neutron stars.
One may also draw information on the deformation of the two stars by tidal effect[5] in their last orbital rotations of before the merger. This provides, for the first time, direct constraints on the internal structure of nuclear matter deep inside neutron stars. Moreover, with the three LIGO-Virgo detectors, one has access to the polarization states of the gravitational wave (which describe the privileged distributions of the orientation of the waves in space-time). This makes it possible to measure the inclination of the binary system orbit with respect to the plane of the sky, an important parameter to be taken into account when confronting with the model of gamma ray bursts (see below): it allows one to test alternative theories of gravity, different from general relativity.
During the inspiraling phase of two neutron stars, the emitted gravitational wave is calculated thanks to the “post-Newtonian” approximation of general relativity. During this phase, the stars velocities are small relative to the speed of light, and the approximation is valid; it has even been shown that the post-Newtonian approximation describes the motion of the two neutron stars and the emitted radiation with an extreme precision. This method has been developed since the 1980’s at the Observatoire de Paris-Meudon and then at the IAP, notably by Luc Blanchet and Guillaume Faye, and has been derived to very high approximation orders.
The results of these calculations are used by the LIGO-Virgo collaboration as a wave “ttemplate”, which provides the “theoretical prediction” for the signal (Figure 3), and leads to the detection of the signal even when it is very weak. The shape of each template is defined by values for the masses and spins of the stars forming the binary system, and each of them are compared to the received signal. In case of agreement between one of the templates and the signal, the parameters of that template are the measured values of the masses and spins. The precision of the post-Newtonian template is crucial, because it must be able to follow with high accuracy the signal will in the frequency band of the detector, that is during approximately 100 seconds and 1500 orbital cycles for GW170817.
Figure 3 : An example of a gravitational wave “template”, describing the variation of the emission of the gravitational wave during the inspiraling phase of two neutron stars, as a function of time (Credit: LIGO-Virgo collaboration).
Finally, a remarkable property of gravitational waves is that one measures directly the distance of the source, estimated here to be 40 megaparsecs, or 130 million light-years. The precision on the measurement of the distance is about 10 megaparsecs, or 32 million light-years. This distance, combined with the redshift measurement of the spectral lines of the host galaxy NGC 4993 (see below), makes it possible to derive an estimate of the value of the Hubble constant, (this is one of the parameters characterizing the expansion of the Universe). The direction of the source, on the other hand, is obtained by the difference in the arrival times of the gravitational wave at the three detectors. Of course, knowing the direction greatly facilitates the search for visible counterparts. The presence of a third interferometer in the network, Virgo, vastly improves the precision in locating the source : it is within a region of the sky of approximately 30 square degrees. Knowing this direction greatly eases the search for the optical counterpart.
The gamma-ray burst
1.7 seconds after the reception of the gravitational waves, the Fermi satellite detects a short gamma-ray burst, that is an emission of photons of energy between a few tens and a few hundred kilo-electron-volts, received in less than two seconds. Figure 4 shows the detected signal.
If the production of a gamma-ray burst following the coalescence of two neutron stars was expected, the observed event exhibits astonishing features: it is intrinsically 100 000 times less luminous than those usually observed, which are at much larger distance, whereas it was emitted at “only” 130 million light-years from Earth. Usually gamma-ray bursts are very energetic and come from a relativistic narrow jet of matter, whose production and properties are still poorly understood. Astrophysicists predict that such a jet may be emitted from a very compact central source (concentrating a large mass within a small radius): a black holeEF="#Ref. 6">[6] surrounded with an accretion disk (matter orbiting the black hole, which gives it shape to the disk), or a rapidly rotating, massive neutron star[6] with an very strong magnetic field. These are precisely the two possibilities considered for the result of the coalescence of two neutron stars, which explains why the emission of the gamma-ray burst in association with the gravitational wave was expected.
In the case of a gamma-ray burst, the relativistic jet propagates in a cone of typical opening angle between 5 and 10 degrees, emitted along the system axis (the axis around which the two stars rotated before coalescence). The low luminosity of the burst that followed the gravitational wave signal suggests that the system was seen “from side”, the main emission having missed the Earth. It should be possible to confirm this scenario from the analysis of the X-ray observations obtained with the Chandra satellite nine days after the burst (whereas no X-ray emission was detected during the first days following the gravitational signal).
Figure 4 : Light curve of GRB 170817A gamma ray burst detected by the Fermi satellite. The peak of the emission in gamma rays (starting at the time marked by the green vertical bar) occurred 1.7 seconds after the coalescence of the two neutron stars: the instant 0 on the horizontal axis corresponds to the instant 0 ofthe coalescence in Figure 2, showing the gravitational signal seen by LIGO.
It should be noted that the delay of 1.7 seconds observed between the gravitational wave signal and the gamma-ray burst provides constrains on the jet “dynamics” (its motions) and the location of the emitted radiation; this is crucial information to distinguish between the different mechanisms proposed to explain the emission of bursts. This work is the field of expertise of another IAP team, led by Frédéric Daigne and Robert Mochkovitch, who is working on physical models of gamma-ray bursts in relation with coalescences since the 1990s. The researchers also contribute to the preparation of the French-Chinese mission “Space Variable Object Monitor” (SVOM) whose objective is to detect numerous bursts in the X-ray and gamma-ray spectral range, and to search for luminous counterparts to the sources detected by the gravitational wave detectors (the launch of SVOM launch is planned for 2021).
The visible and near infrared emission
The optical counterpart of the “transient” (ie ephemeral emission) is detected as soon as it gets dark, that is about ten hours after the first alert. It is discovered independently, and during the same night, by several groups conducting supernova[7] searches in South America. initially names SSS17a by the group observing in Las Campanas in Chile, it was subsequently labelled AT2017gfo according to the official nomenclature of the International Astronomical Union (AT for astronomical transient, 2017 for the year; it is the 4950th transient detected in 2017).
The NGC 4993 galaxy, in which this remarkable new object is located, is a standard lenticular galaxy with a rather old stellar population. This is not a contradiction, because a long period of time (a billion years or more) can elapse between the formation of the two neutron stars (as a result of the explosion of two massive stars rapidly evolving into Supernovae) and their coalescence: they initially approach very slowly one another.
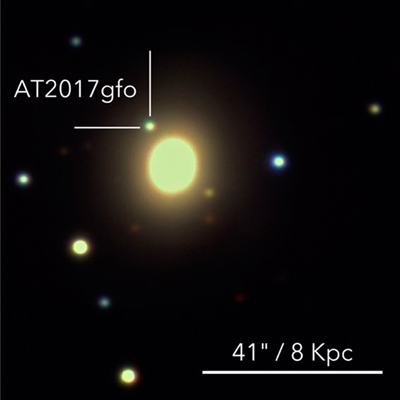
To understand the nature of this new source, its discovery was immediately followed by a series of photometric observations (astronomical images in various filters), and spectra acquisition[8], which lasted about ten days. These observations show an object whose luminosity decreases rapidl with time until it becomes undetectable (Figure 6), and whose colour shifts progressively from blue to red (and near-infrared). This evolution is in perfect agreement with the theoretical predictions for a kilonova[1]. Just after the coalescence of the two neutrons stars, which destroys them, neutron-rich matter is expelled at high speed. The physical conditions are then favourable to very specific nuclear reactions: very heavy nuclei are formed by fast capture of neutrons. Many of these newly formed nuclei are radioactive: they heat the ejected material which cools by radiating; this perfectly explains the intensity and colour evolution of the kilonova.
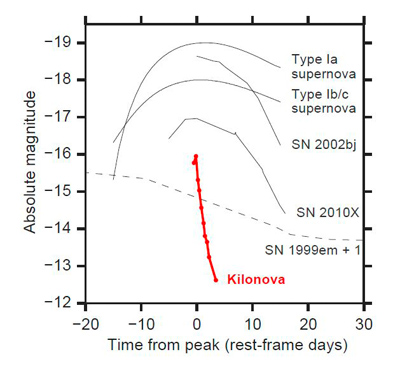
Some of the very first spectra were taken with the ESO 3.5 meter New Technology Telescope (NTT) and the 8.2 meter Very Large Telescope (VLT) in Chile, as part of the European “Public ESO Spectroscopic Survey of Transient Objects” (PESSTO, http://www.pessto.org ; programme under the responsibility of S. J. Smartt from the Queen’s University in Belfast). IAP observers have been involved since its beginning in 2012 (M.Dennefeld, and PhD student J. Palmerio). These observations agree perfectly with the theoretical predictions: the kilonova spectrum is close to a black body[9] with an initial temperature exceeding 10,000 degrees (when the object is blue), and then decreases rapidly in a few days, the object becoming redder. In the spectrum obtained two and a half day after the explosion, one can identify absorptionA HREF="#Ref. 10">[10] lines from two heavy elements never seen before, Cesium and Tellurium, both heavier than iron. This confirms the scenario of nucleosynthesis (synthesis of atomic nuclei) of heavy elements by rapid capture of neutrons. More generally, the detailed modeling of the light curve and spectra of the kilonova in its late phases shows that the ejected matter has a high opacity (ability of a medium to absorb the light that passes through it): this indicates the presence of nuclei from the lanthanides[11] group even heavier than Tellurium or Cesium.
It seems therefore clear that the expected steps of nucleosynthesis have taken place, and have probably been carried out until the formation of the heaviest elements like gold, platinum, or even uranium. The origin of these rare elements, in the Universe, has long been mysterious. Another IAP team (Elisabeth Vangioni and Frederic Daigne) has been working since 2015 on the scenario of formation of these heavy elements during the coalescence of neutron stars, and the August event brings strong arguments in favour of these models.
Multi-messenger astronomy
The August 17 event is remarkable in several ways. On one hand, the impressive predictive power of physics is verified, as such an association between gravitational waves, a gamma-ray burst and a kilonova had been predicted for a long time. On the other hand, confirming such an association has required an unprecedented international coordination between instrumental developments and observation campaigns. With this event, a new branch of astronomy is born, “multi-messenger” astronomy : it illustrates the large complementarity that exists between the gravitational messenger which makes it possible to finely characterize the binary neutron stars system just before the coalescence, and the luminous messenger which informs us on the results of this coalescence. At last, new answers have been brought to the difficult question of the origin of the heaviest atomic elements in the Universe.
The fact that this detection occurred after the second data acquisition from LIGO-Virgo, shortly after the Virgo interferometer has joined the two interferometers LIGO, suggests that it will be followed by other discoveries in the coming years. This will make it possible to answer new questions, such as the frequency of these events, the nature of the compact object that forms after coalescence, the geometry of relativistic ejection. It is an adventure that is just beginning, and to which IAP researchers will continue to take part.
Footnotes
[1] The name of kilonova comes from the fact that its brightness peak is about one thousand times larger than for a nova, an explosive phenomenon observed at the surface of some white dwarf stars (kilo is the multiplicative by 1000). In comparison, a supernova can be one million times brighter than a nova.[2] In astronomy, a binary system is composed of two objects of the Universe linked by the gravitational force. The objects follow a bound orbit around their common center of mass.
[3] A neutron star is the remnant of the core of a massive star having exploded in supernova; it is mainly made of neutrons. It is an object of extreme density as it has a typical mass of 1.4 times that of the Sun but the size of a big city (a radius of about 10 km). What prevents the star from collapsing under its own weight is the huge pressure resulting from the strong interaction between neutrons, the only one capable to counterbalance gravitation in such a dense stellar object. A neutron star is barely observable, except if it produces a pulsed radio emission (it is then a “pulsar”), or if it is surrounded by an accretion disk made of matter stripped from a companion star: it then manifests itself as powerful X-ray source (an “X-ray binary”).
[4] The preliminary analysis of the gravitational signal published by LIGO-Virgo makes assumptions on the spin of the neutron stars, whereas a more sophisticated analysis should make it possible to measure the spins.
[5] Tidal forces are the result of the different gravitational attraction that applies to the different parts of a body as a function of distance.They are for example responsible for the deformation of the height of ocean caused by the Moon attraction at the surface of the Earth.
[6] A black hole is the final state of the collape, due to the gravitational force, of a massive star at the end of its evolution. The full mass is then concentrated within a point called a “singularity”, and from which no light can escape when emitted from below a frontier called the “horizon”.
[7] Supernovae are very bright luminous phenomena resulting from stellar explosions: thermonuclear explosion of a white dwarf for type Ia supernovae; explosions of massive stars following the gravitational collapse of their central core for the other types, which specifically produce neutron stars. Many sky surveys look for supernovae: they are rare phenomena, as there is about one supernova per century in a galaxy like the Milky Way. To collect the largest possible sample is necessary for a better understanding of the explosion mechanism(s). Moreover, in the case of type Ia supernovae, one can obtain their distance from the combination of their apparent brightness and colour, which allows them to be used as tools for cosmology. It is supernovae which made it possible to discover the acceleration of the expansion of the Universe.
[8] A spectrum is the light distribution emitted by an object that is dispersed by a prism (or equivalent device), and which makes it possible to measure the amount of light emitted by an object according to the wavelengths (or colour of the light).
[9] A black body is an ideal object that would perfectly absorb all the electromagnetic energy it receives, without reflecting or transmitting it.
[10] Emission or absorption lines are excess or lack of light observed in the spectra of astronomical objects, at specific wavelengths corresponding to chemical elements present in the object.
[11] Lanthanides are the generic name given to nuclei of higher atomic masses than Iron (A=56), starting with Lanthane (A=57).
Links

https://journals.aps.org/prl/abstract/10.1103/PhysRevLett.119.161101 (version publique)

http://iopscience.iop.org/article/10.3847/2041-8213/aa91c9 (version publique)

https://www.nature.com/nature/journal/vaop/ncurrent/full/nature24291.html (version publique)

https://www.nature.com/nature/journal/vaap/ncurrent/full/nature24303.html (version publique)




Note: A conference (in French) open to the public, organised by IAP and UPMC will be held by Frédéric Daigne, January 9, 2018, at Farabeuf Amphitheater (15 rue de l'école de médecine - 75006 Paris): « Première détection d’une fusion d’étoiles à neutrons en ondes gravitationnelles et de la lumière associée : la naissance d’une nouvelle astronomie à plusieurs messagers ».
Practical informations: http://www.iap.fr/science/conferences/conferences.php?annee=2018
Writing and contacts
- Luc Blanchet
Institut d’astrophysique de Paris, CNRS, UPMC
luc [dot]blanchet [at] iap [dot] fr
- Frédéric Daigne
Institut d’astrophysique de Paris, CNRS, UPMC
frédéric [dot]daigne [at] iap [dot] fr
- Michel Dennefeld
Institut d’astrophysique de Paris, CNRS, UPMC
michel [dot]dennefeld [at] iap [dot] fr
- Robert Mochkovitch
Institut d’astrophysique de Paris, CNRS, UPMC
robert [dot]mochkovitch [at] iap [dot] fr
Web writing: Valérie de Lapparent
Layout: Jean Mouette
October 2017